The Neutrino Puzzle
22 March 2014 | By
Having explored the latest results on what we call 'heavy flavour' or physics of particles containing a b-quark (see The Penguin Domination by Jessica Levêque), we embarked on a much lighter subject: neutrinos.
It was as if a fresh breeze swept through the audience. Partly because we are surrounded by snow-capped mountains but mostly because of the topic -- neutrino physics has been bubbling with activity these past few years. Many new measurements were shown, adding several pieces to the neutrino puzzle. But we are still far from having a clear idea of the picture we are trying to build, piece by piece.
Neutrinos are special particles. They are at the heart of some of the most exciting fundamental problems that particle physicists are trying to solve. But neutrinos are elusive, a characteristic that makes it difficult to study them. Physicists must use their ingenuity to compete at developing new kinds of detectors capable of measuring neutrinos coming from different sources.
There are a few things we know about neutrinos. In the Standard Model, neutrinos are neutral leptons that were thought to be massless. There are three neutrino species -- electron, muon and tau neutrinos, each associated to the other three leptons in the Model -- electron, muon and tau. They are the second most common particle in the universe after photon but are not well-known to the public. They interact with matter through weak interaction which makes them difficult to catch. But physicists like challenges and build experiments to detect and measure the flux of neutrinos coming from sources outside of our solar system or the sun, through the atmosphere, produced by terrestrial nuclear power plants or particle accelerators.
Most of these experiments were only sensitive to one neutrino species and at first, all these measurements appeared to be inconsistent. The picture got clearer when the Super Kamiokande experiment in Japan established in 1998 that neutrinos can oscillate from one species to another. Which means that an electron-neutrino can transform itself into a muon-neutrino and vice-versa. This explains, for instance, why the solar electron-neutrino measured flux is well below the one predicted by the solar model -- because a fraction of them oscillate into muon-neutrinos that were not detected. The important consequence of the oscillation is that it can only occur if neutrinos have mass!
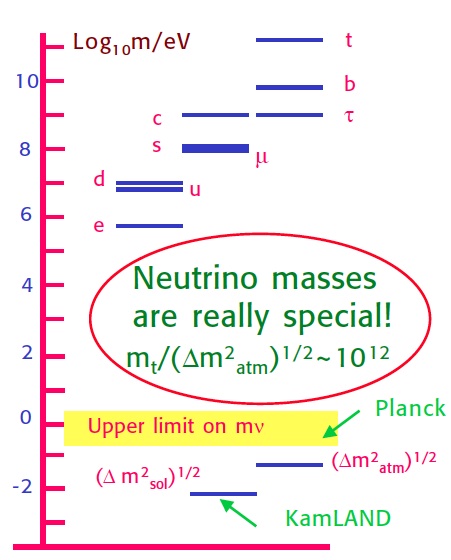
Since then, new experiments have been built to measure the probability of oscillation between different neutrino species and infer a measurement of their mass. At the conference, several measurements of these parameters were shown and we now know with fair precision the different oscillation probabilities as well as the mass differences between neutrino species. However, we still don’t know the mass itself although cosmological experiments allow us to set an upper limit on the sum of the masses of the three neutrino species, which is below an eV (electronVolt). Moreover, new experimental inconsistencies appear: some experiments do not observe the expected number of neutrinos, even with the oscillations taken into account.
So now, new questions have arisen: Where does the neutrino mass come from? Why is it so far from the other lepton masses? As it is massive and weakly interacting, could the neutrino be part of the dark matter of the universe? Is the neutrino its own anti-particle? Are there more than three neutrinos? Where are the high energetic neutrinos coming from?
Some experiments like IceCube are now able to map neutrinos coming from the universe and this is like doing astronomy with neutrinos!
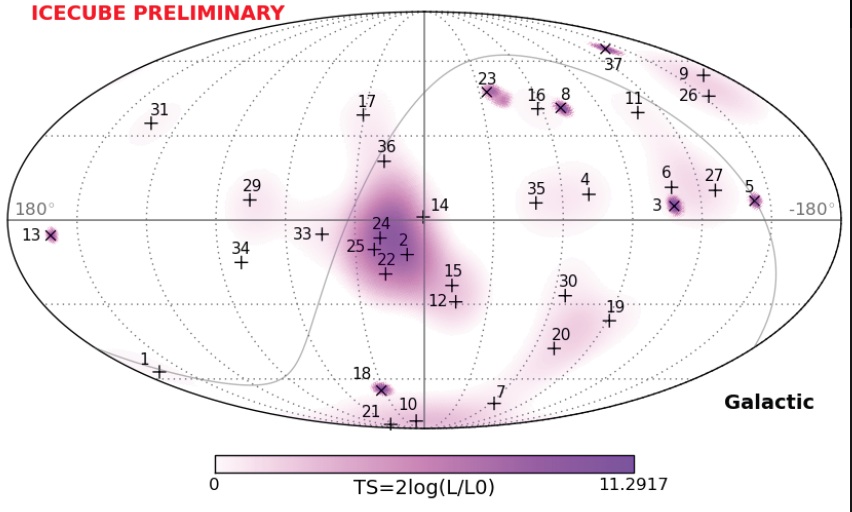
During the session, several theoreticians proposed models that try to conciliate the different observations and answers to the above questions: Couldn’t there be a new species of neutrino in which the others could oscillate? Is the neutrino description in the standard model complete: couldn’t they have (as the other leptons) right-handed partners? This last option is interesting since it could explain why the standard neutrino mass is so small and perhaps also part of the universe dark matter as the right handed-neutrinos could be very massive.
Theoretical talks alternated with experimental ones describing future experiments that are currently being developed to help solve the puzzle. These experiments are being built by smaller collaborations in comparison to the LHC teams. The experiments can be located in the South Pole to take advantage of the ice as an interacting medium for the detector or in the depth of a disused mine to fight efficiently against cosmic ray background. The proposed technologies are also very different depending on the aim of the measurement but all experiments need a very low and well-controlled background, as the number of observed neutrinos is always small.
Stay tuned! There is no doubt that new results on neutrinos will come soon but in the meantime, my colleagues and I will catch some fresh air during a long lunch break up on the snowy mountains. After all, it is important to rest our brains in order to prepare for presentations of the top quark, the Higgs boson and other new results from the LHC in the next sessions.
So, what does a particle physicist, with her brain at rest, see in the surrounding mountains?
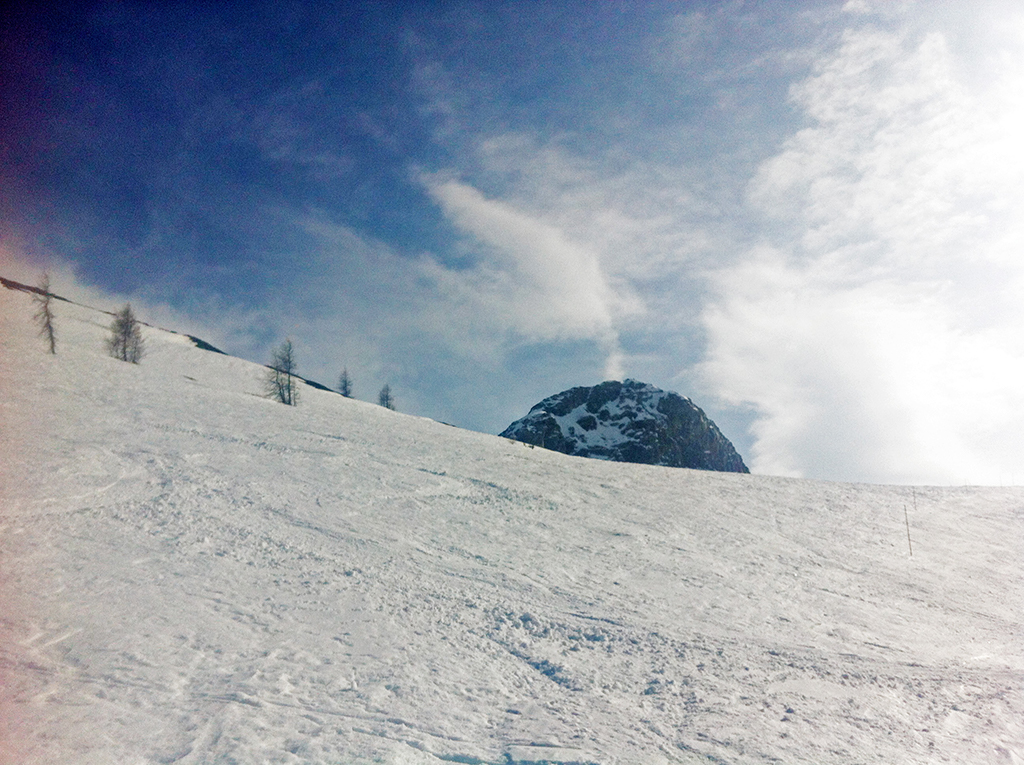
Well...

The Higgs boson, of course!!!